Stainless steel is a vital material in numerous industries, prized for its durability, corrosion resistance, and aesthetic appeal. However, not all stainless steel is created equal. Among its many classifications, austenitic and martensitic stainless steels stand out as two of the most prominent and versatile types. Understanding the differences between these stainless steels is critical for selecting the right material for specific applications, whether it’s for industrial machinery, medical instruments, or culinary tools. This article will explore the key distinctions between austenitic and martensitic stainless steel, their unique properties, and how these attributes determine their diverse applications. By the end, you’ll have a deeper insight into how material composition influences performance, helping you make more informed decisions for your projects.
What is Martensitic Stainless Steel?
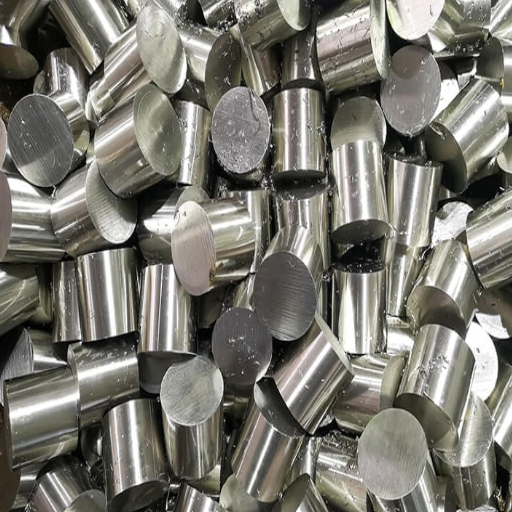
Understanding the Microstructure of Martensitic Steel
Martensitic stainless steel exhibits a body-centered tetragonal (BCT) crystal structure, which results from rapid cooling, or quenching, from high temperatures. This microstructure is unique to martensitic steel and is responsible for its high hardness and strength. The achievement of martensitic transformation becomes possible at lower temperatures than austenitic transformation, where carbon atoms become imprisoned within the BCT lattice during cooling, which is considered as enhancing internal stresses aids towards the mechanical properties of the material.
The microstructure of martensitic stainless steel is also characterized by its lower chromium content, between 12 to 18 percent, along with differing quantities of carbon. These characteristics enable martensitic steel to sustain extreme levels of hardness and resistance to abrasion, making it suitable for applications demanding great durability under mechanical stress. On the downside, these characteristics often lead to a reduction in stainless steel’s resistance to corrosion compared to other types of stainless steel, like austenitic steels.
The microstructure and properties of martensitic stainless steel are greatly influenced by heat treatment. For example, the tempering process after quenching achieves stress relief and carbon redistribution in the steel’s microstructure, mitigating brittleness and enhancing toughness. This controllable balance of properties is one reason why martensitic stainless steel is extensively used in cutting tools, turbine blades, and other materials and parts that require high strength and versatility.
Applications and Hardness of Martensitic Grades
Applications that require high strength and durability greatly benefit from martensitic stainless steels. Their propensity to achieve these properties from heat treatments, including quenching and tempering, makes them suitable for manufacturing surgical instruments, knife blades, and other cutting tools. They also maintain a durable backbone in high-stress environments, including use in industrial machinery, turbine blades, and automotive components.
The hardness of the alloy varies depending on the heat treatment and composition. In general, they perform within the range of 40 to 65 HRC on the Rockwell Hardness Scale due to alloying with carbon and other elements and selective thermal processes. The increased hardness is due to the grains which consist of martensite, a powerful solid state solution which improves structural integrity and abrasion resistance, dominating the microstructure of the alloys.
Despite the benefits, it is still common to find restrictions between hardness and toughness. It is a common practice to temper the material after the initial quenching to relieve internal stress and balance mechanical properties to reduce brittleness while maintaining adequate hardness. Such combinations of properties, along with reliable performance across a variety of applications, make martensitic stainless steels suitable for numerous industrial needs.
The Role of Carbon Content in Martensitic Steel
The mechanical properties and performance level of martensitic stainless steels heavily rely on additional factors like their carbon content. Carbon’s main impact is through the formation of martensite during the steel quenching process, which increases its strength and hardness. Carbon does enhance the hardness of the steel, making it suitable for applications that require surface durability and wear resistance. However, increased carbon causes higher brittleness, which reduces toughness.
Carbon also affects the corrosion resistance and machinability of the steel. Martensitic stainless steels have a fair level of corrosion resistance, but higher carbon content decreases this quality due to a lack of chromium to form protective oxide layers. A different situation occurs with lower carbon levels since those increase oxidation resistance, thereby making the steel more useful in mechanically demanding yet oxidizing conditions.
The specific use case for each steel type influences the carbon content, which in turn is centered around the usage. For tools that require high cutting prowess, such as blades, surgical tools, and turbine blades, cutting tools should contain more carbon (around 0.3 to 1.0%). On the other hand, parts with tougher structure and high resistance to wear and tear should have lower levels of carbon. Adjusting the levels of carbon in steel types unlocks the control over performance and durability.
Exploring Austenitic Stainless Steel
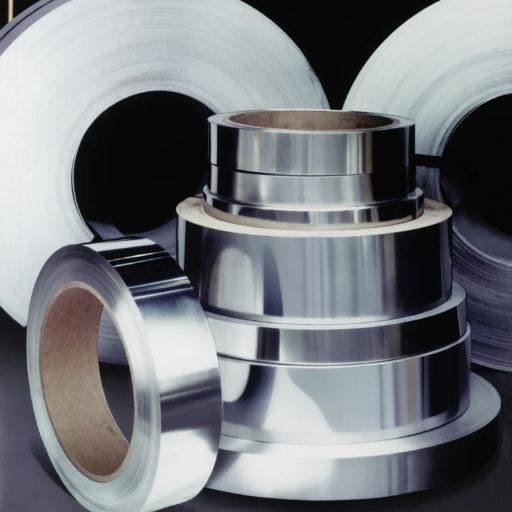
Characteristics of Austenitic Stainless Steel
Austenitic Stainless Steel is the most profusely used type of stainless steel due to its qualities such as high corrosion resistance, and high levels of mechanical properties at varying temperatures. This steel is distinguished by having a high chromium (between 16% and 26%) and nickel (6% to 22%) content. The results turn into a face-centered cubic (FCC) crystal structure. The face-centered cubic microstructure also provides astonishing levels of ductility, toughness, and non-magnetic characteristics when annealed.
Below the melting point of iron, austenitic stainless steel has high non-magnetic characteristics, and astonishing nonoxidation and wear resistance, making FCC stainless steel assured to be used in hostile environments. Marine applications or processing chemicals harshly would need the use of such materials. Certain grades like 316 and 316L have earned great reputations for resisting chloride pitting, which is immensely important in trying conditions with liquids containing salt or chloride. Furthermore, the L grade (for example, 304L, 316L) also reduces the chances of carbide precipitation causing intergranular corrosion while still maintaining mechanical strengths.
This family of alloys is also highly formable and weldable, enabling it to be manufactured into intricate components without loss of strength. Because of the high toughness of austenitic stainless steel, it can be used in cryogenic applications. On the other hand, austenitic grades tend to be costlier than other types of stainless steel, like ferritic or martensitic, due to their relatively high nickel content.
It should be mentioned that work hardening is a distinguishing feature of austenitic stainless steel. Mechanically, the material undergoes a significant strengthening due to strain hardening, which makes it suitable for more demanding applications like industrial machinery and kitchen devices that require greater durability and wear resistance.
Corrosion Resistance in Austenitic Stainless Steel
The major alloying element used in Austenitic stainless steel is chromium, ranging from 16% to 26%. This provides the steel with excellent corrosion resistance owing to self-passivation. The live or self-healing oxide film formed is highly effective in humid or water mediums, thus cables made of the alloy can be used in chemical processing equipment or marine structures.
Moreover, the oxide film formed also protects the gear against further corrosional attack, thus making it suitable to work in versatile conditions. This is because the nickel in these grade alloys increases their stability and resistance to aggressive chemicals like acid or chlorides. To mention one example, molybdenum is used in grades like 316 to disable pitting and crevice erosion alongside venturing into chloride-rich settings or seawater. Mentioned standard grades of Austenitic alloys are also known for their strength and resistance to intergranular erosion in heat treatment, and low carbon variants like 304L.
The high-temperature and oxidative resistance of austenitic stainless steel is attributed to its microstructure. Its versatility shines through during thermal processing applications and furnace components due to scaling and oxidation resistance at elevated temperatures. Enhanced mechanical and corrosion resistance maintains reliability for critical applications while decreasing maintenance costs and extending material life.
Common Applications for Austenitic Steel
Austenitic stainless steel is an essential material in numerous industries because of its remarkable mechanical properties, its ability to resist high temperatures, and its protection against oxidation and corrosion. Five applications that use austenitic stainless steel are listed below:
- Chemical Processing Equipment: Because of its vast application in the chemical industry, austenitic stainless steel is one of the most durable stainless steel grades. 316 or 316L grades of austenitic stainless steel are applied in the manufacturing of reaction vessels, heat exchangers, and storage tanks owing to their high corrosion resistance.
- Food and Beverage Industry: The non-reactive properties of austenitic stainless steel makes it a prime material for hygienic use in the food and beverage industry. 304 and 316 grades stainless austenitic steel are used for food processing machinery as well as storage containers and kitchen equipment.
- Medical Devices and Surgical Instruments: Implants, scalpels, and forceps are surgical steel instruments that use austenitic stainless steel because it is biocompatible, non-corrosive, and mechanically stable. 316L grade or surgical steel is commonly used for implants due to its corrosion resistance to body fluids.
- Components of Automotive and Aerospace Industries: Used in the construction of vessels, turbochargers, and aircraft parts, automotive exhaust systems utilize high-strength, heat-resistant austenitic stainless steel, as grades 321 and 347 endure thermal fatigue along with chronic exhaust strain.
- Building and Architectural Structures Materials: Combined with being aesthetically appealing, austenitic stainless steel is highly sought after for construction purposes, making it ideal for clad buildings and structural reinforcements, as it is known to withstand weathering. It can be noted in the modern skyscrapers and bridges built using grade 304, which serves for enduring performance and structural support.
Each of these applications highlights the versatility and reliability of austenitic stainless steel in demanding industries and environments.
Difference Between Austenitic and Martensitic Stainless Steel
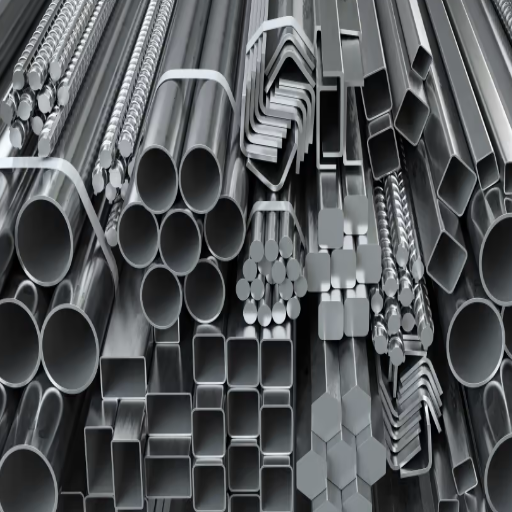
Crystalline Structure and Its Impact
The crystalline structure of stainless steels largely determines their properties, effectiveness, and use in certain applications. Stainless steels are divided into two main classes based on their crystal structure: austenitic and martensitic. These two classes have different mechanical and chemical behaviors, which stem from their structure. The major differences in crystalline structure, along with their consequences, can be summarized in the following key points:
- Austenitic Structure (Face-Centered Cubic – FCC): The crystal structure of austenitic stainless steel is face-centered cubic (FCC). This crystalline structure enables ductility and toughness in steel. Furthermore, the FCC structure provides corrosion resistance, which is beneficial in conditions where moisture, chemicals, or extreme temperatures prevail. In addition to these factors, the FCC allows stainless steel to maintain its non-magnetic properties, which is useful for some devices that require electromagnetic neutrality.
- Martensitic Structure (Body-Centered Tetragonal – BCT): The most distinguishing feature of martensitic stainless steel is its body-centered tetragonal (BCT) structure which occurs when the parent austenite transforms at low temperatures. This crystal orientation offers greater hardness and strength, but with lower corrosion resistance in comparison to austenitic grades. This inferior resistance comes from the increased presence of carbon in martensitic steels, which aids in the development of this structure. Such properties make Martensitic steels suitable for cutlery, surgical, and other tools.
- Grain Size Impact: The mechanical characteristics of a material, such as its toughness and tensile strength, are affected by the size of its grains in the crystal lattice. Smaller grain size is known to enhance these characteristics, which in turn, increases wear resistance. Austenitic stainless steels are known to control the grain size with greater finesse during manufacturing, which makes them perform better in precision applications.
- Deformation Behavior: Welding and forming processes become simpler due to the greater plastic deformation of austenitic stainless steel, which has an FCC structure. The more accommodating BCT structure of martensitic stainless steel requires control to avoid brittleness and cracking due to its less accommodating nature.
- Thermal Expansion and Conductivity: Thermal properties are most directly affected by the crystalline structures. While martensitic steel has high thermal conductivity, austenitic steel has an FCC structure, which gives it lower thermal conductivity. Expansion and contraction of materials are essential for structures such as thermal insulation systems and heat exchangers; other alternatives or material stability are also required.
Stainless steel grades that are used for certain industrial and operational uses can be precisely selected with attention paid to the impact of crystalline structure on the properties.
Comparing Corrosion Resistance and Toughness
Two key properties of stainless steel, its corrosion resistance and toughness, are influenced by its alloy composition and microstructure. For example, austenitic stainless steels have high chromium and nickel contents, which bestows them with marvelous corrosion resistance. These metals are subjected to oxidative and pitting corrosion, but because of the passive oxide layer that forms on the surface, the material is protected. Hence, they are appropriate for use in stringent conditions such as those in the medical, marine, and chemical processing industries, which expose materials to corrosive media for extended periods.
Martensitic stainless steels carry with them a higher carbon content which results in superior mechanical toughness at the expense of corrosion resistance. In aggressive environments, these steels become more prone to localized corrosion due to the formation of carbides in martensitic steels which reduce the amount of chromium available to be transformed into protective oxide layer.
The austenitic and ferritic phases of duplex steels grant them toughness and corrosion resistance, thus achieving a balance. The mixed microstructure enhances smoothness, strength, and resistance to stress corrosion cracking, enabling these steels to perform effectively in the oil and gas industry, where there is significant environmental and mechanical wear.
Recognizing the properties and how each grade of stainless steel responds to different environmental and mechanical stresses helps engineers tailor specific solutions to meet industrial needs while balancing the material’s performance, longevity, and expense.
Understanding Heat Treatment Differences
Heat treatment processes are important in modifying the mechanical and physical characteristics of stainless steel for certain industrial uses. The main treatments include annealing, quenching, tempering, and stress relieve, and each process does something different. As an example, the goal of annealing is to soften material, relieve internal stress, and improve ductility that was created during fabrication. The process of annealing requires heating stainless steel to a temperature range of 1,900 – 2,100 degrees (1,038 to 1,149) and followed by cooling at a controlled rate.
Martensitic stainless steels require an optimal combination of toughness and hardness, this is achieved through quenching and tempering. The process of quenching is rapid cooling of the material often in water or oil and traps carbon atoms within the crystalline structure which increases hardness. The next step, tempering, reduces brittleness by reheating the material to a lower temperature, transformation of the microstructure happens.
Another step of critical importance to the heat treatment is stress relieving. Austenitic stainless steels are susceptible to stress corrosion cracking when under high tensile stress. This involves heating the material to around750 – 1500 degrees, 400 to 815 degrees, which depends on the alloy. The focus of stress relieving is on reducing residual stress without damaging the mechanical properties of the material.
The development of modern technologies such as computer-controlled furnaces, real-time monitoring, and automation has simplified processes and maximized the performance of materials in harsh industrial settings. A steel’s ability to endure components fulfills the criteria regarding strength, corrosion resistance, and the operational lifespan is dictated by the meticulous selection and application of heat treatment procedures, including the use of stainless steel.
How Does Ferritic Stainless Steel Compare?
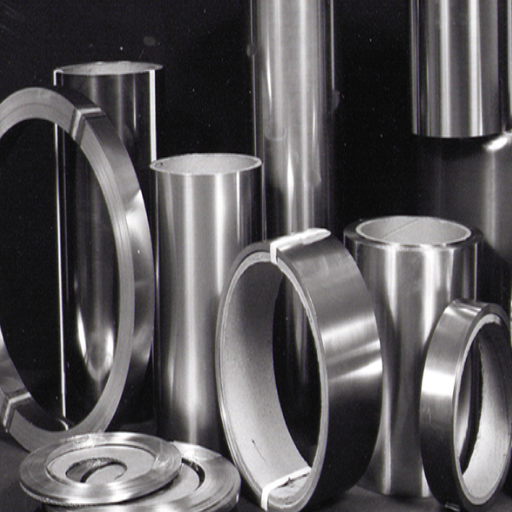
Characteristics and Applications of Ferritic Steel
Ferritic stainless steels have a primary composition of iron and chromium and typically contain between 10.5 to 30% of chromium with a low amount of carbon, usually less than 0.1%. This makes composition enables ferritic steels to resist stress corrosion cracking, along with possessing good thermal conductivity and lethally exceptional oxidation resistance. Unlike austenitic grades, ferritic stainless steels have magnetic properties due to their body-centered cubic (BCC) crystal structure. Ferritic steels also have lower thermal expansion, making them suitable for high-temperature applications.
One of the notable properties is its superior performance against chlorine compounds, which makes it perfect for marine applications where salt or acidic substances are prevalent. Along with that, ferritic steels have better fabrication properties than high-strength alloys, easing the forming and welding processes on structural components. Because of their balanced case effectiveness and corrosion resistance, grades 430 and 409 are widely used in automotive exhaust systems and heat exchangers in addition to architectural applications.
The scalability of ferritic stainless steels makes them fit for use in a variety of industries. As a case in point, type 444 is frequently used for water heater tanks and plumbing fixtures because of unmatched resistance to pitting and crevice corrosion. Unlike austenitic steels, ferritic steels have limited applicatios, but their lower price and their high-performance characteristics make them ideal for specialized industrial applications.
Comparing Ferritic vs Austenitic and Martensitic
Crystal structure, corrosion resistance, strength as well as application use differentiate ferritic, austenitic, and martensitic stainless steels.
Aspect |
Ferritic |
Austenitic |
Martensitic |
---|---|---|---|
Structure |
BCC |
FCC |
BCC (quenched) |
Rust Resistance |
Moderate |
High |
Moderate |
Hardness |
Moderate |
Low to Moderate |
High |
Flexibility |
High |
High |
Low |
Magnetism |
Yes |
No |
Yes |
Heat Treatment |
No |
No |
Yes |
Applications |
Automotive, Heat Exchangers |
Cookware, Chemical Equip. |
Cutlery, Surgical Tools |
Chromium Level |
11-30% |
16-25% |
11.5-18% |
Nickel Level |
None |
8-14% |
None |
Heat Conductivity |
Moderate |
Low |
Moderate |
Challenges in Welding Austenitic and Martensitic Stainless Steel
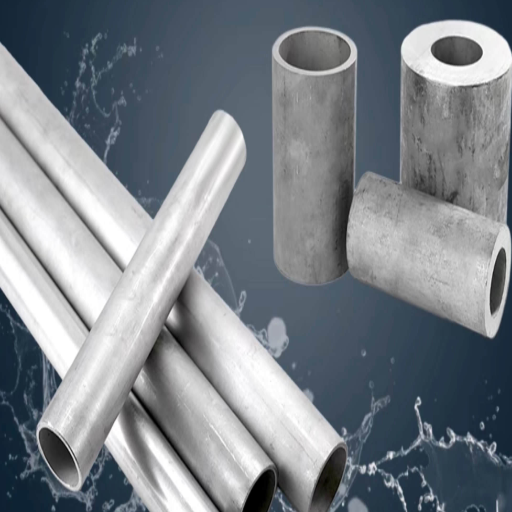
Addressing Cracking and Distortion during Welding
Cracking and distortion are two problems of utmost importance and are encountered during welding due to the thermal stress and unwanted welding, as well as improper welding. Effective dealing with these issues requires understanding the extensive body of knowledge of the material and mechanical factors, as well as using specific techniques concerning the material, welding, and the process itself. Below are five methods for dealing with these problems:
- Preheating the Material: Pre-heating minimizes the weld and its gap that will always exist between the weld area and the corresponding base metal. Thus, lowering thermal stress at higher temperatures. Take, for instance, for high carbon steels, keeping a preheat value of somewhere between 200°F and 400°F (93°C to 204°C) may dramatically lower the chances of hydrogen-controlled cold cracking.
- Optimizing Heat Input: The control of thermal input is a delicate procedure as contrived preheating/fusion alone leads to melting. Excessively Higher Input is able to result in distortion and unsaturated heating leads to the resultant lacking sufficient fusion. For Low-alloy steels, keeping the input of heat to levels between 2.0-2.5 kJ/mm results in both, sufficient dosage and minimal distortion.
- Using Proper Filler Materials: Appropriate selection of filler materials can increase the chances of uncontrolled remains for stress and, therefore, cracking. An instance is welding dissimilar metals and using filler materials having erg (ERNiCrMo-3) for nickel-based alloys helps as it has a thermally competent range of composition and expansion.
- Managing Weld Sequence: An optimally planned welding sequence helps reduce distortion. Methods such as backstepping and skip welding allow for an even distribution of heat, therefore lessening warping. For instance, systematic skip welding on thin sheets of stainless steel results in minimal distortion.
- Post Weld Heat Treatment (PWHT): The mechanical properties and residual stresses of a weldment are improved when undergoing PWHT. Stress-relieving comes from controlled cooling after heating martensitic stainless steels at 1100°F to 1200°F (593°C to 649°C) to reduce cracking and enhance integrity.
Through these efforts alongside monitoring the process, weld quality can greatly improve, and common defects such as cracking and distortion greatly reduced.
Techniques for Welding Different Steel Grades
In solving the problem of weldability of different grades of steel, my starting point is the detailed study of the alloy compositions and their properties. For ferritic stainless steels, such as Type 409 or 444, controlled heat input is the most important. These steels tend to grain coarsen in the heat-affected zone (HAZ), which will reduce mechanical properties. Because of this, I control the heat to low levels, restrict temperature rise between passes in multi-pass welding to a predetermined limit, and control temperature to ensure that optimal weld integrity is maintained. For ferritic steels, post-weld heat treatments are almost always unnecessary, however, light stabilization annealing might be used if some changes are needed for dimension control.
When welding, the focus on thermal contraction and expansion is of high priority for austenitic stainless steels. This group of materials includes 304 and 316, which also possess ferritic steel and have much higher thermal expansion coefficients. Therefore, increasing the chances of warping or generating stress opens up. I practically always use back purging with inert gas, either argon or helium, to keep the chromium oxide layer, which protects the stainless steel from oxidizing and corrosive substances. Besides, for the same reason, I tend to use ER308L as filler material because of its intermediate composition, which reduces the chance of sensitization or intergranular corrosion in high-temperature places.
Because of their hardenability, martensitic stainless steels present a distinct set of challenges. For example, Type 410 and 420 steels require preheating before welding to minimize the potential for cracking in the heat-affected zone. To combat the effects of excessive hardness and brittleness, I maintain a controlled cooling rate after welding. A martensitic structure is weakened by residual stress, so post-weld heat treatment is essential to temper (restore toughness) that structure and relieve stresses. The strategies I implement differ based on the specific grade of stainless steel, however, the controllable variables of heat input, filler material, and post-weld procedures are fundamental to the desired outcome.
References
- Austenitic stainless steels – Academia.edu
- Effect of Strain Rate on Tensile Properties of Austenitic Stainless Steels at Room Temperature – Colorado School of Mines
- The influence of martensitic transformation on the formability of 304L stainless steel sheet – Massachusetts Institute of Technology (MIT)
Frequently Asked Questions (FAQ)
Q: What is the primary difference between austenitic and martensitic stainless steel?
A: The primary difference lies in their metallurgical structure and composition. Austenitic stainless steels contain higher levels of chromium and nickel, which give them better corrosion resistance and ductility. Martensitic stainless steels, on the other hand, have higher carbon content and can be heat-treated for increased hardness and strength, but tend to have limited corrosion resistance.
Q: How does the chemical composition of austenitic stainless steel compare to martensitic stainless steel?
A: Austenitic stainless steels typically have higher chromium and nickel content compared to martensitic stainless steels, which contain more carbon and less nickel. This difference in chemical composition results in varied physical properties and applications.
Q: Why are austenitic stainless steels considered easier to weld compared to martensitic types?
A: Austenitic stainless steels, such as those in the 300 series, have a higher chromium and nickel content, which makes them more ductile and easier to weld. Martensitic stainless steels, with their higher carbon content, are more prone to cracking during welding, making them more difficult to weld.
Q: What are some common applications of austenitic stainless steels?
A: Austenitic stainless steels, such as 304H, 317L, and 321H, are commonly used in applications requiring excellent corrosion resistance and formability, such as kitchen equipment, chemical processing equipment, and architectural components.
Q: Can martensitic stainless steels be heat-treated for improved performance?
A: Yes, martensitic stainless steels can be heat-treated to enhance their hardness and strength. This makes them suitable for applications like cutlery, surgical instruments, and turbine blades where high strength is required.
Q: How does the presence of ferrite affect the properties of stainless steels?
A: The presence of ferrite in stainless steels can affect their magnetic properties and corrosion resistance. While austenitic stainless steels have a non-magnetic cubic form, the addition of ferrite can enhance strength but might reduce the corrosion resistance.
Q: What are the challenges associated with welding martensitic stainless steels?
A: Martensitic stainless steels are challenging to weld due to their high carbon content, which can lead to embrittlement and cracking. Preheating and post-weld heat treatment are often required to mitigate these issues.
Q: What distinguishes the 300 series of austenitic stainless steels from the 400 series?
A: The 300 series, including types like 303, 304H, and 310, is austenitic and known for its excellent corrosion resistance and ease of fabrication. The 400 series, containing martensitic and ferritic grades, generally offers higher strength but lower corrosion resistance compared to the austenitic counterparts.
Q: Are there any austenitic stainless steels that provide resistance to high temperatures?
A: Yes, grades such as 309S, 310, and 310S are designed for high-temperature applications. These steels maintain strength and resist oxidation in elevated temperatures, making them suitable for furnace parts and heat exchangers.
Q: What role does manganese play in the composition of stainless steels?
A: Manganese can replace some of the nickel in stainless steels, providing similar corrosion resistance while reducing cost. It also enhances strength and hardness. However, excessive manganese can lead to embrittlement in certain types of steel.